Mahdiyeh Hasani
Department of Food Science, University of Guelph, Guelph, Ontario, Canada
Keith Warriner
Department of Food Science, University of Guelph, Guelph, Ontario, Canada
The first foodborne illness case linked to fresh produce likely occurred way before the first Caesar salad was prepared back in 1924. However, it was not until the 1970s that incidence of foodborne illness outbreaks linked to fresh produce were documented (Keith Warriner, Huber, Namvar, Fan & Dunfield, 2009). From that time, the number of cases increased year-on-year, and fresh produce is the current leading cause of foodborne illness outbreaks (Gutierrez-Rodriguez & Adhikari, 2018). Several events that occurred in the 1990s placed fresh produce in the food safety spotlight. Specifically, the evolution of pathogen detection methods had been improved, along with epidemiology and investigative resources.
However, the major contributory factors were the increased availability of fresh produce, introduction of the bagged salad, vacuum hydrocooling and global distribution networks (Hu et al., 2019; H.P. Li, Tajkarimi & Osburn, 2008). Additionally, if one considers leafy greens, then it is possible for contamination to be acquired in the field that can then be spread across different batches during processing. Given that most fresh produce is consumed raw, any contamination acquired in the field, transportation or processing is carried through to the end consumer.
When washing doesn’t cut it
To address the food safety issues linked to fresh produce there was an increased focus on post-harvest washing to remove contamination acquired during crop production and processing (Kayla Murray, Wu, Shi, Jun Xue & Warriner, 2017). To this end, there have been numerous publications on different sanitizers for washing fresh produce (Beuchat, 1995; Kayla Murray et al., 2017). Yet, it was evident that promising results from laboratory trials were not translating to decontamination efficacy during commercial processing. It was initially thought that internalization of pathogens such as Escherichia coli O157:H7 was the primary reason for the lack of decontamination efficacy by post-harvest washing (Erickson et al., 2010). Although this may have contributed to protecting pathogens from sanitizers, it soon became apparent much more significant issues were at play.
Essentially, when produce is washed, the organic and inorganic constituents released into the water would sequester free chlorine, thereby neutralizing a high proportion of antimicrobial activity (K. Murray, Aldossari, Wu & Warriner, 2018). This was compounded by the fact that oxidation reduction potential (ORP) monitoring of wash tanks was proven ineffective as a means of indirectly quantifying freechlorine in wash tanks.
In 2012, there was accumulating evidence from different research groups that wash water – rather than decontaminating produce – was causing cross-contamination (Barrera, Blenkinsop & Warriner, 2012). Indeed, several high-profile outbreaks linked to fresh produce could be linked to lack of control of the sanitizer concentrations in the post-harvest wash tanks (Kayla Murray et al., 2017). Research also was performed to demonstrate maintaining adequate sanitizer concentrations could minimize cross-contamination, although not totally reliable at removing pathogens from produce (Kayla Murray et al., 2017).
To some processors, this was enough, as it only required increasing the concentration of sanitizer in wash tanks. However, with the advent of the Food Safety Modernization Act (FSMA) and Safe Foods for Canadians Act (SFCA) it was a requirement that processors implement pathogen validated and monitored (Gombas, 2019). Therefore, effective alternatives to post-harvest washing were required for decontaminating fresh produce that were commercially feasible, cost-effective and accepted by consumers.
Advanced oxidation process (AOP)
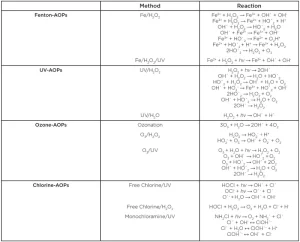
The origins of AOP can be traced back to 1883, when H.J. Fenton discovered that tartaric acid could be oxidized by hydrogen peroxide with Fe2+ acting as the catalyst (Giannakis, 2019). There remains a debate over the mechanism of the Fenton reaction, with the generation of oxidized ferric ions being the active agent vs. generation of hydroxyl radicals derived from hydrogen peroxide (Table 1). The reason for the uncertainty over the reaction mechanism is due to the transient nature of the free-radical species that is the order of milliseconds. This is positive in one respect that there are no lasting residues, although on the counter it means that the radicals are required to be generated in-situ (i.e. on the surface to be decontaminated).
The early use of the Fenton reaction was as an analytical reagent in oxidation reactions (Fenton-Reagent). It was not until the 1960s that the Fenton reaction found utility in wastewater treatment whereby pesticides, along with other pollutants, could be readily degraded by the addition of hydrogen peroxide and ferric ions (Alaton, Balcioglu & Bahnemann, 2002). The efficacy of the Fenton reaction was demonstrated to be significantly improved by the simultaneous use of UV light in a process referred to as Photo-Fenton reaction. The reaction is thought to photo-oxidize the ferric ion, thereby continuing the hydroxyl radical formation (Table 1).
Although the Photo-Fenton reaction is highly efficient, there are disadvantages with respect to requiring pH < 3 to prevent the precipitation of ferric compounds, cost of Fe2+ salts, along with leaving iron residues that can potentially enhance bacterial biofilm formation (Kiilerich, Brejnrod, Vollertsen & Kiilerich, 2019). This led to the development of UV hydrogen peroxide-based process that, although less efficient at producing hydroxyl radicals than the Fenton reaction, does not require the addition of ferric ions (Hermosilla, Merayo, Gasco & Blanco, 2015).
AOP for surface decontamination
The use of AOP for water treatment is well established and remains an active area of research. In comparison, the use of AOP for surface decontamination has received less attention. An AOP-based treatment for surface disinfection was developed by the packaging company ELOPAK for decontaminating the interior of cartons used in ultra-hightemperature (UHT) beverage processes (Bayliss & Waites, 1979; K. Warriner et al., 2000). The process was devised from the works of Bayliss and Waites, who demonstrated > 5 log reduction of Bacillus endospores by using hydrogen peroxide spray of 1% and 30-second UV-C treatment (Bayliss & Waites, 1979). The researchers also demonstrated that using excess hydrogen peroxide (>2%) reduced the lethality of the process by resulting in neutralization of the hydroxyl radicals through termination reactions (Bayliss & Waites, 1979).
The incentive to apply AOP to packaging sterilization was partly to avoid patent infringement by the process operated by Tetra Pak, which applied 35% hydrogen peroxide in carton packaging decontamination. There were significant benefits of using AOP over hydrogen peroxide alone. Specifically, low-hydrogen-peroxide solutions could be applied that represent significant cost saving and reduced risk of carrying over residues. The commercial AOP could be used to treat 150 cartons/minute and was thereby compatible with high throughput aseptic filling machines (Ansari & Datta, 2003).
AOP for decontaminating fresh produce
The convergence of food safety issues linked to fresh produce with the advent of AOP for surface decontamination resulted in a line of research to assess the efficacy of the process as an intervention step. A treatment chamber based on spraying peroxide on produce whilst under constant illumination by UV-C (254 nm) was assessed for inactivating human pathogens (E. coli O157:H7; Salmonella) and spoilage bacteria (Pectobacterium, Pseudomonas) introduced on and within a range of fresh produce (Hadjok, Mittal & Warriner, 2008). It was found that a treatment using 30-second UV-C, 1.5% hydrogen peroxide at 50°C resulted in > 4 log cfu eduction of Salmonella on and within shredded lettuce. It was found that using hydrogen peroxide or UV alone supported 1 to 2 log cfu reduction, as did applying the AOP at 20°C compared to 50°C (Hadjok et al., 2008). This demonstrated that using a combination of UV-C and peroxide at 50°C provided synergistic decontamination efficiency.
When the same treatment was applied to other produce types, comparable log reduction of pathogens and spoilage bacteria were obtained with leafy greens, cauliflower and broccoli. Interestingly, the lowest log count reductions were obtained with tomatoes, despite having a smoother surface compared to the other produce tested (Hadjok et al., 2008). Yet in all cases, the AOP outperformed standard hypochlorite postharvest washes. Additional studies demonstrated the AOP to inactivate MS2 bacteriophages (surrogate for norovirus) on lettuce with no changes in the visual appearance of the leafy green during storage (Xie, Hajdok, Mittal & Warriner, 2008). The result was confi rmed by Li et al. (2011) who demonstrated a combination of hydrogen peroxide vapor (2.5%) and UV-C supported a > 4 log reduction of norovirus surrogate on lettuce, in addition to stainless steel surfaces (D. Li et al., 2011).
Optimization of AOP by response surface methodology
Response surface methodology (RSM) is a statistical method for determining the response derived from multiple operating parameters (Kaushik, Rao, & Mishra, 2017). The main advantage of RSM is that a relatively small number of experiments need to be performed compared to the conventional approach of assessing the effect of a single variable at a time (Bezerra, Santelli, Oliveira, Villar & Escaleira, 2008; Takashina, Leifeld, Zelinski, Mafra & Igarashi-Mafra, 2018). In the context of AOP, the independent variables are UV-C dose, intensity and hydrogen peroxide concentration. The dependent variables are the log reduction of the target microbe and changes in the sensory quality of the fresh produce being treated.

RSM was applied to devising the commercial AOP treatment for treating lemons (Hasani, et al., 2019). Here, 20 treatment combinations were selected based on varying the UV dose, intensity and hydrogen peroxide treatment with the log reduction of Aspergillus niger spores and degree of browning representing the dependent variables. From the data generated it was possible to generate surface response plots that graphically represent the contribution of each of the independent variables (Figure 1). From the plots it can be observed that the hydrogen
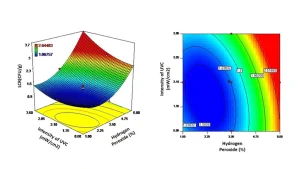
peroxide concentration is a key contributor, along with UV-C intensity, in both defining the log reduction (Figure 1) and browning of lemons (Figure 2). By using the surface response plots, it is possible to identify the optimal treatment for maximizing the antimicrobial activity without negatively affecting lemon color. In the current example, the optimized treatment was 1.88% v/v hydrogen peroxide with a UV-C intensity of 3.26 mW/cm2 and 20s treatment time.
The commercialization of AOP for fresh produce decontamination
Although the AOP system demonstrated potential as an alternative to post-harvest washing of fresh produce, there was little interest to commercialize the technology in 2008. However, this changed with an outbreak of Listeria monocytogenes linked to caramel apples (Buchanan, Gorris, Hayman, Jackson & Whiting, 2017). The actual outbreak was caused by an apple processor located in California where the fruit was contaminated in the orchard and the pathogen spread in dunk tank water. When the stick was inserted into the apple in preparing the candy apple, the Listeria was introduced into the center, thereby damaging the tissue that supported growth of the pathogen (K. Murray, Moyer, Wu, Goyette & Warriner, 2018). In response to the outbreak, it was proposed to refrigerate candy apples to minimize the growth of L. monocytogenes. However, this would only delay the growth of the pathogen by two to three days. Based on the risk associated with candy apples, many retailers withdrew the product from sale, collapsing the whole market and resulting in significant economic losses to processors who were not implicated in the outbreak.
To regain the confidence of retailers to restart marketing candy apples again, a more effective decontamination method for apples beyond washing was required. To this end, the AOP approach was revisited and studies undertaken to assess efficacy at inactivating L. monocytogenes on the surface and within whole apples. In this example, a combination of UV-C, hydrogen peroxide and ozone (Table 1) was applied on apples inoculated on the surface along with vacuum infiltrated into the stem scar tissue. A treatment using 54 mJ/cm2 (30s treatment time), 6% hydrogen peroxide and 2g/h ozone supported a 3 log reduction of L. monocytogenes both on the surface and internal structures of apples (K. Murray, Moyer, et al., 2018). The reactor used in the trial was based on a conveyer system with hydrogen peroxide spray heads that the apples passed through before entering an array of UV-C and ozone-producing lamps. In this case, the ozone provided an additional source of hydroxyl radicals through interacting with the hydrogen peroxide and via decomposition via the UV-C light.
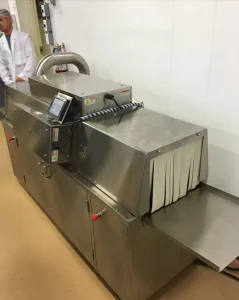
The laboratory scale unit was further developed into a functional commercial AOP reactor that housed four ozone generating lamps (185 nm) and six UV-C lamps (Figure 3). The additional ozone-generating lamps enabled the hydrogen peroxide concentration to be decreased to 3% without loss of antimicrobial efficacy. When the treatment was used to decontaminate other produce types it was found that Listeria on tomatoes was reduced by 1.2 log cfu compared to 5 log cfu on cantaloupes, avocados, peaches and lemons (Figure 4).
The results would be counterintuitive given that tomatoes have a relatively smooth surface compared to cantaloupes, which are heavily netted and uneven. Although yet to be studied in detail, a possible explanation is that the uneven surface traps a greater
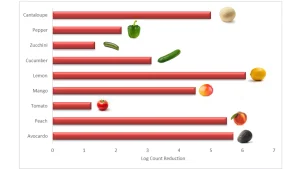
quantity of hydrogen peroxide that can be converted to hydroxyl radicals. In contrast, a smoother surface, such as found on tomatoes and zucchini, retains less of the hydrogen peroxide spray when entering the UV chamber. Supporting evidence for the theory resides back to the short half-life of hydroxyl radicals that must be generated at the required site on the surface of produce. It can be envisaged that a more successful treatment for produce, such as tomatoes, could be achieved by applying the hydrogen peroxide as a spray while simultaneously being exposed to ozone and UV-C.
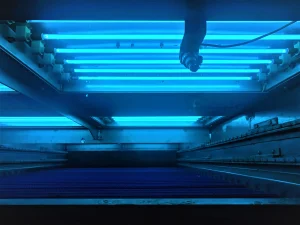
The largest AOP unit has been installed recently at a citrus fruit processor as a microbial (pathogen and spoilage microbes) reduction step (Figure 5). The unit is 10 meters long and consists of hydrogen peroxide spray bars, four modules housing UV-C and ozone-generating lamps. The unit can decontaminate 4,000 lemons per hour. That underlines the ability to scale approach to any size of operation.
Next generation of AOP reactors for surface decontamination
The AOP has previously been confined primarily to wastewater treatment, although it has been demonstrated to have strong potential as a surface disinfection method. From a commercial perspective, the treatment can be readily installed into existing processing to provide a continuous process that rapidly decontaminates surfaces with minimal water usage.
One disadvantage of the process is the need for hydrogen peroxide, which – even when used at low concentrations – still required large volumes to be applied over a typical processing period, depending on the capacity of the line. In addition, the conversion of hydrogen peroxide to hydroxyl radicals via the UV-C mediated degradation is relatively inefficient, given the low absorption coefficient at 254 nm (Furatian & Mohseni, 2018). Although gaseous ozone can contribute a source of hydroxyl radicals, this too can be relatively inefficient, given that the radicals are generated in the headspace of the chamber rather than on the produce surface.
Alternatives to hydrogen peroxide, such as chlorine dioxide gas, have illustrated synergistic antimicrobial activity for inaction of pathogens on spinach (Park, Kang & Kang, 2018). A treatment of 10 ppmv chlorine dioxide with constant UV illumination supported a > 5 log cfu reduction of Salmonella on spinach with treatment time of 20 minutes, suggesting a lower radical generation (Park et al., 2018). Authors did not suggest any interaction between UV and chlorine dioxide, although one could envision either generation of radicals and/or increase sensitivity of target cells.
Chlorine in the form of hypochlorous acid also could serve as a precursor for generating chloride radicals that have a longer half-life compared to the hydroxyl radical and also absorb more strongly at 254 nm, hence are more efficient (Furatian & Mohseni, 2018). In the UV-chlorine AOP system, the hypochlorous acid can be introduced as hypochlorite but would require the pH to be adjusted to five to six to ensure low levels of the non-reactive hypochlorite anion. Consequently, the hypochlorite would need to be acidified, which would require the addition of acid in combination with hardware to monitor pH. A more convenient method to produce the hypochlorous acid would be through electrolysis of sodium chloride solutions, commonly termed electrolyzed water (Graca, Abadias, Salazar & Nunes, 2011). Here, sodium chloride (i.e. common salt) is introduced into an electrolysis cell, whereby hypochlorous acid is generated at the anode and sodium hydroxide at the cathode. The hypochlorous acid fraction then can be fed into the AOP system to generate the chloride radical with the byproduct of free chlorine that contributes additional antimicrobial activity (Table 1). The disadvantage of the electrolysis approach is the need for additional hardware, although the cost of electrolysis cells is decreasing and so would be less expensive compared to hydrogen peroxide.
In addition to radical sources, the introduction of different wavelengths for supporting photo-AOP can be envisaged. To date, the use of UV-C at 254 nm has been historically applied in AOP due to the availability of lamps. However, with the advent of LEDs the applied wavelength to facilitate the photooxidation of hydrogen peroxide can be optimized, thereby increasing the efficacy of hydroxyl radical generation. It has been reported that 260 nm supported the highest degradation of anatoxin-a (cyanotoxin) in an AOP process compared to 270, 280 and 290 nm (Matafonova & Batoev, 2018; Verma & Sillanpaa, 2015). It was proposed that, at 260 nm, there was an increase in hydroxyl radical formation and increased susceptibility of the anatoxin-a to oxidation, thereby enhancing the reaction kinetics. The use of LEDs for surface disinfection by AOP has yet to be explored, but the benefits in fabricating novel compact reactors for use in domestic and industrial applications can be anticipated.
Conclusions
To date, AOP has been focused on wastewater treatment with surface decontamination being restricted to carton packaging. More recent research has extended the approach to decontaminate fresh produce, with commercial scale units becoming available. The key to commercialization of the AOP technology was identifying a need derived from, and increased focus on, food safety due to outbreaks and/or introduction of new regulations. Although the current AOP process is effective, it can be further improved by using alternative radical sources, such as hypochlorous acid and LED light sources. In addition, although the current focus of AOP is in the surface decontamination of fresh produce, it can be extended to other commodity groups along with food contact surfaces.
References
Alaton, I. A., Balcioglu, I. A., & Bahnemann, D. W. (2002). Advanced oxidation of a reactive dyebath effluent: comparison of O-3, H2O2/UV-C and TiO2/UV-A processes. Water Research, 36(5), 1143-1154.
Ansari, M. I. A., & Datta, A. K. (2003). An overview of sterilization methods for packaging materials used in aseptic packaging systems. Food and Bioproducts Processing, 81(C1), 57-65.
Barrera, M. J., Blenkinsop, R., & Warriner, K. (2012). The effect of different processing parameters on the efficacy of commercial post-harvest washing of minimally processed spinach and shredded lettuce. Food Control, 25(2). 745-751.
Bayliss, C. E., & Waites, W. M. (1979). The combined effect of hydrogen peroxide and ultraviolet irradiation on bacterial spores. Journal of Applied Microbiology, 47, 263-269.
Beuchat, L. R. (1995). Present and emerging control measures for fresh-cut packaged vegetables.
Bezerra, M. A., Santelli, R. E., Oliveira, E. P., Villar, L. S., & Escaleira, L. A. (2008). Response surface methodology (RSM) as a tool for optimization in analytical chemistry. Talanta, 76(5), 965-977.
Buchanan, R. L., Gorris, L. G. M., Hayman, M. M., Jackson, T. C., & Whiting, R. C. (2017). A review of Listeria monocytogenes: An update on outbreaks, virulence, dose-response, ecology, and risk assessments. Food Control, 75, 1-13.
Erickson, M. C., Liao, J., Payton, A. S., Riley, D. G., Webb, C. C., Davey, L. E., . . . Beuchat, L. R. (2010). Preharvest Internalization of Escherichia coli O157:H7 into Lettuce Leaves, as Affected by Insect and Physical Damage. Journal of Food Protection, 73(10), 1809-1816.
Furatian, L., & Mohseni, M. (2018). Influence of chloride on the 185 nm advanced oxidation process. Chemosphere, 199, 263-268.
Giannakis, S. (2019). A review of the concepts, recent advances and niche applications of the (photo) Fenton process, beyond water/wastewater treatment: Surface functionalization, biomass treatment, combatting cancer and other medical uses. Applied Catalysis B-Environmental, 248, 309-319.
Gombas, K. (2019). Introduction to the Food Safety Modernization Act. Cereal Foods World, 64(2).
Graca, A., Abadias, M., Salazar, M., & Nunes, C. (2011). The use of electrolyzed water as a disinfectant for minimally processed apples. Postharvest Biology and Technology, 61(2-3), 172-177.
Gutierrez-Rodriguez, E., & Adhikari, A. (2018). Preharvest Farming Practices Impacting Fresh Produce Safety. Microbiology Spectrum, 6(2).
Hadjok, C., Mittal, G. S., & Warriner, K. (2008). Inactivation of human pathogens and spoilage bacteria on the surface and internalized within fresh produce by using a combination of ultraviolet light and hydrogen peroxide. Journal of Applied Microbiology, 104(4), 1014-1024.
Hasani, M., Chudyk, J., Murray, K., Lim, L-T, Lubitz, D & Warriner K. (2019). Inactivation of Salmonella, Listeria monocytogenes, Aspergillus and Penicillium on Lemons using Advanced Oxidation Process Optimized through Response Surface Methodology. Innovative Food Science & Emerging Technologies (In Press). Available at https://www.sciencedirect.com/science/article/pii/S1466856419301341?via%3Dihub
Hermosilla, D., Merayo, N., Gasco, A., & Blanco, A. (2015). The application of advanced oxidation technologies to the treatment of effluents from the pulp and paper industry: a review. Environmental Science,168-191.
Hu, K., Liu, J., Li, B., Liu, L. L., Gharibzahedi, S. M. T., Su, Y., . . . Guo, Y. (2019). Global research trends in food safety in agriculture and industry from 1991 to 2018: A data-driven analysis. Trends in Food Science & Technology, 85, 262-276.
Kaushik, N., Rao, P. S., & Mishra, H. N. (2017). Effect of high pressure and thermal processing on spoilage-causing enzymes in mango (Mangifera indica). Food Research International, 100, 885-893.
Kiilerich, B., Brejnrod, A. D., Vollertsen, J., & Kiilerich, P. (2019). Variations in microbiome composition of sewer biofilms due to ferrous and ferric iron dosing. Cogent Environmental Science, 5(1).
Li, D., Baert, L., De Jonghe, M., Van Coillie, E., Ryckeboer, J., Devlieghere, F., & Uyttendaele, M. (2011). Inactivation of Murine Norovirus 1, Coliphage phi X174, and Bacillus fragilis Phage B40-8 on Surfaces and Fresh-Cut Iceberg Lettuce by Hydrogen Peroxide and UV Light. Applied and Environmental Microbiology, 77(4), 1399-1404.
Li, H. P., Tajkarimi, M., & Osburn, B. I. (2008). Impact of vacuum cooling on Escherichia coli O157 : H7 infiltration into lettuce tissue. Applied and Environmental Microbiology, 74(10), 3138-3142.
Matafonova, G., & Batoev, V. (2018). Recent advances in application of UV light-emitting diodes for degrading organic pollutants in water through advanced oxidation processes: A review. Water Research, 132, 177-189.
Murray, K., Aldossari, H., Wu, F., & Warriner, K. (2018). Dynamic changes in free-chlorine levels within a commercial post-harvest wash and prevention of cross-contamination between shredded lettuce batches. Food Control, 85, 127-134.
Murray, K., Moyer, P., Wu, F., Goyette, J. B., & Warriner, K. (2018). Inactivation of Listeria monocytogenes on and within Apples Destined for Caramel Apple Production by Using Sequential Forced Air Ozone Gas Followed by a Continuous Advanced Oxidative Process Treatment. Journal of food protection, 81, 357-364.
Murray, K., Wu, F., Shi, J., Jun Xue, S., & Warriner, K. (2017). Challenges in the microbiological food safety of fresh produce: Limitations of post-harvest washing and the need for alternative interventions. Food Quality and Safety, 1, 289-301.
Park, S. H., Kang, J. W., & Kang, D. H. (2018). Inactivation of foodborne pathogens on fresh produce by combined treatment with UV-C radiation and chlorine dioxide gas, and mechanisms of synergistic inactivation. Food Control, 92, 331-340.
Takashina, T. A., Leifeld, V., Zelinski, D. W., Mafra, M. R., & Igarashi-Mafra, L. (2018). Application of Response Surface Methodology for Coffee Effluent Treatment by Ozone and Combined Ozone/UV. Ozone-Science & Engineering, 40(4), 293-304.
Verma, S., & Sillanpaa, M. (2015). Degradation of anatoxin-a by UV-C LED and UV-C LED/H2O2 advanced oxidation processes. Chemical Engineering Journal, 274, 274-281.
von Sonntag, C. (2008). Advanced oxidation processes: mechanistic aspects. Water Science and Technology, 58(5), 1015-1021.
Warriner, K., Huber, A., Namvar, A., Fan, W., & Dunfield, K. (2009). Recent Advances in the Microbial Safety of Fresh Fruits and Vegetables. In S. L. Taylor (Ed.), Advances in Food and Nutrition Research, Vol 57 (Vol. 57, pp. 155-208): Elsevier Academic Press Inc, 525 B Street, Suite 1900, San Diego, Ca 92101-4495 USA.
Warriner, K., Rysstad, G., Murden, A., Rumsby, P., Thomas, D., & Waites, W. M. (2000). Inactivation of Bacillus subtilis spores on packaging surfaces by u.v. excimer laser irradiation. Journal of Applied Microbiology, 88(4), 678-685.
Xie, Y., Hajdok, C., Mittal, G. S., & Warriner, K. (2008). Inactivation of MS2 F (+) coliphage on lettuce by a combination of UV light and hydrogen peroxide. Journal of food protection, 71, 903-907.
Contact: kwarrine@uoguelph.ca