By Pankaj Chowdhury, Application Scientist, and Siva R. Sarathy, UV Applications Manager, Trojan Technologies
Municipal wastewater can contain harmful chemicals and pathogens, so removing or reducing these substances is desirable to protect environmental and public health. Decontaminating municipal wastewater for drinking water reuse is an effective strategy to address water shortages in areas affected by droughts or rapid urbanization.1 Organic chemicals in reclaimed water often are higher than in traditional drinking water sources. 2 These chemicals can include industrial chemicals, pesticides, cyanotoxins, hormones, pharmaceuticals, poly- and perfluoroalkyl substances (PFAS), disinfection byproducts (DBPs) and DBP precursors. 3 Other compounds can be present in reclaimed water at concentrations that could pose a chronic health risk, yet there are no standards or guidelines for them. 4
This highlights the need for further attention and consideration to ensure the safety of reclaimed water for public health. Transforming secondary or tertiary wastewater effluents into safe, potable water requires an advanced purification process. This involves a sequence of robust barriers to eliminate chemical contaminants and pathogens effectively. These barriers include microfiltration (MF) or ultrafiltration (UF), reverse osmosis (RO) and an advanced UV light-based oxidation process (UV AOP). This multi-stage purification approach ensures that the resulting water meets the highest quality standards for potable reuse. 5
Direct UV Photolysis
Advanced treatment processes are implemented to eliminate trace organic contaminants, such as pharmaceuticals, N-nitrosodimethylamine (NDMA) and pesticides that may pass through RO membranes to some extent. 5 The most common advanced treatment process includes UV AOP or ozonation for simultaneous disinfection and removal of micropollutants. Degradation mainly occurs through oxidation reactions with non-selective hydroxyl radicals. 6
The rejection of NDMA by RO membranes varies significantly, ranging from 8% to 82%, depending on the membrane and operating conditions. 7 Direct UV photolysis is highly effective in reducing NDMA levels using high energy. To effectively break down high concentrations of NDMA using photolysis, a significantly greater amount of energy is required than the energy needed for disinfecting typical drinking water sources. 8
When UV is employed, 254 nm light produced by low-pressure mercury amalgam lamps effectively can degrade pharmaceuticals by direct photolysis but at much greater UV doses (i.e., several thousand mJ/cm2) compared to those typically used for disinfection (up to 80 mJ/cm2). 6
Parameters Affecting Direct UV Photolysis
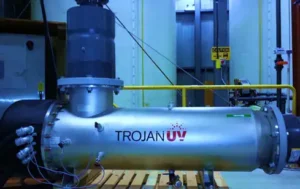
The degradation of a compound when exposed to UV-C light is influenced heavily by the compound’s molecular structure and absorption spectrum. Essential photochemical parameters, such as the molar extinction coefficient (ε) and quantum yield (QY), play a crucial role in determining degradation rates. Furthermore, the pH of water is a critical factor, as it alters throughout the potable reuse treatment process. For instance, the pH of permeate water from reverse osmosis, ranging from 5.5 to 6.5, significantly can impact the photochemical properties of various pharmaceuticals in the mixture, leading to changes in their UV responses.
Many researchers have studied the impact of pH on the direct UV photolysis of halonitromethanes, sulfa drugs, tetracycline antibiotics, analgesics and fluoroquinolone. Most recently, the authors performed direct UV photolysis experiments at different pH with NDMA and 10 pharmaceuticals – sulfathiazole (STZ), sulfisoxazole (SFX), sulfamethoxazole (SMX), ciprofloxacin (CIP), fluoxetine (FLX), diclofenac (DCF), antipyrine (AP), chlortetracycline (CTC), chloramphenicol (CAP) and hydrochlorothiazide (HCT) – using a full-scale UV photoreactor (Figure 1) with 30 low-pressure UV lamps by varying water flow rates (375–2700 L/min1) and UV fluences (400–4000 mJ/cm2). 6
pH-Dependent Photochemical Properties
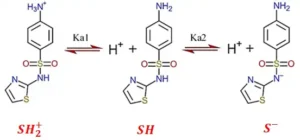
Depending on pH and acid dissociation constant (pKa), e and QY are susceptible to change. However, not many studies have been found on this topic. pH is a crucial factor, as the pH of the water changes at different stages of the potable reuse treatment train. Solutions’ pH values could change a compound’s protonation state and absorption spectrum. Figures 2 and 3 show sulfathiazole’s ionization and absorption spectra between pH 5 and 8.
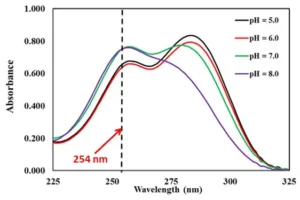
The authors’ investigation assessed the molar extinction coefficients of NDMA and 10 pharmaceuticals at 254 nm in buffered Milli-Q water at pH levels of 5, 7 and 8 (Figure 4). The findings revealed significant variations in molar extinction coefficients for all three sulfa drugs (STZ, SFX, SMX) and CIP in response to changes in pH. Conversely, apart from HCT, the remaining compounds exhibited minimal changes in molar extinction coefficients, less than 2%, across the tested pH range.
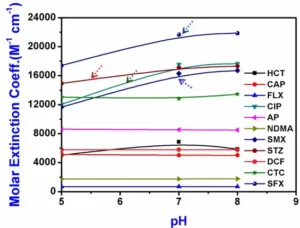
When the pH exceeds the second pKa value, sulfa drugs primarily exist in an anionic form. In this state, the sulfinol-nitrogen atom gains two lone pairs of electrons and engages in p-π conjugation with sulfonyl and penta-heterocycle groups. The resulting strong repulsion between the two lone pairs increases the energy difference between the HOMO (highest occupied molecular orbital) and LUMO (lowest unoccupied molecular orbital), causing a blue shift in the UV–Visible absorption spectra and higher absorbance for the anions. 9
In a mixture where different ionic forms coexist for specific compounds, e and QY (f) can be calculated from e and f, and the distribution coefficient of each form in the mixture (Equations 1 and 2) where (+), (o) and (–) notations express the molar extinction coefficients and quantum yields for cationic, neutral and anionic forms of the organic compound, respectively.


Equation 3 presents the log reduction of a compound because of degradation by UV photolysis, where C0 is the initial concentration in mg/ L, and D is the UV dose in mJ/cm2. The log reduction can be calculated by knowing the pH, CpH and D values under a specific operating condition. 6

Full-Scale Direct UV Photolysis
In this work, 6 the authors meticulously analyzed the photochemical properties of specific pharmaceuticals. The findings were applied to validate the performance of a full-scale photoreactor using a sophisticated model. The research delved into the photolysis kinetics of NDMA and 10 pharmaceuticals, employing a state-of-the-art collimated beam apparatus with an LP-UV lamp. Furthermore, a process model was introduced that integrates a Computational Fluid Dynamics (CFD)-based UV fluence in the photoreactor with a photolysis kinetics model. This model encompasses the measurement of pH-dependent molar absorbance and quantum yield to accurately determine the log reduction in the reactor.
Full-scale tests were conducted at pH 6.2, where eight experiments were carried out at various UV fluences ranging from 0 to 4000 mJ/cm2. This broad range of UV fluence was achieved by adjusting the feed flow rate and ballast power level. The photochemical properties of each compound at pH 6.2 were calculated. In the mixture, the direct UV photolysis of 11 compounds followed pseudo-first-order kinetics. The photolysis rates of the compounds followed the order: SFX>CTC>DCF>STZ >SMX>NDMA>AP>CIP>FLX>CAP>HCT.
In full-scale tests, Equation 3, together with the Lagrangian UV dose model derived from CFD, was used to evaluate the log reduction of the model compounds. The log reduction of sulfamethoxazole was higher than NDMA, whereas the log reductions of sulfathiazole, antipyrine, fluoxetine, chloramphenicol and hydrochlorothiazide were lower than NDMA at pH 6.2. The observed degradation trend followed that of the calculated H90 (mJ/cm2) values (UV fluence for 1 log reduction), which had the same order of SMX <NDMA < STZ < AP < FLX < CAP < HCT at pH 6.2.
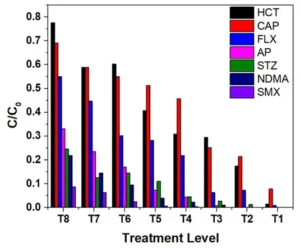
Figure 5 shows the normalized concentration (C/C0) vs. treatment level plot of six pharmaceuticals and NDMA at treatment levels T1 to T8 (T1 is the highest treatment level, and T8 is the lowest treatment level). A Lagrangian UV dose model was used for each test condition to predict the delivered UV dose at the given flow rate, UVT and ballast power level. The best predictions were obtained for FLX, CAP, AP and NDMA, with an excellent match occurring at a lower level of treatment. The missing data points at T1, T2 and T3 were due to non-detectable residual concentrations of the compounds above 2.5 log reduction.
Interpretations
The experimental log reductions of pharmaceuticals under varying UV treatment conditions, with doses ranging from 0 to 4000 mJ/cm2, were compared with predictions using a novel pH-dependent photochemical degradation model. This model links QY with the degree of dissociation of ionic species and the dose model developed in this study. Sulfa drugs, diclofenac and NDMA exhibited high removal via UV photolysis, but they necessitated a significantly higher UV dose than that required for standard water disinfection. This underscores the importance of additional treatments for water recycling.
Pankaj Chowdhury, application scientist for Trojan Technologies, holds a Ph.D. in Chemical Engineering from Western University, as well as an undergraduate degree in Chemistry and Chemical Engineering and a master’s degree in chemical engineering from the University of Calcutta, India. Currently, he is the Water Testing Services team leader at Trojan Technologies, where he provides analytical services across all sectors of the business, including municipal UV, industrial UV, residential UV and filtration. He can be reached at pchowdhury@trojantechnologies.com.
Siva R. Sarathy, UV applications manager for Trojan Technologies, received a Bachelor’s degree in Environmental Engineering from the University of Waterloo and a Ph.D. in Chemical Engineering from the University of British Columbia. He has 20 years of experience in water and wastewater treatment processes, with the last 15 years as a scientist with Trojan Technologies. He currently leads a team of application scientists and engineers that provides specialized technical services, pre-sales and post-sales, for Trojan’s customers. He can be reached at ssarathy@trojantechnologies.com.
References
- U.S. Environmental Protection Agency (EPA) 2017. Potable Reuse Compendium (EPA/810/R-17/002).
- Nguyen, T., Westerhoff, P., Furlong, E.T., Kolpin, D.W., Batt, A.L., Mash, H.E., Schenck, K.M., Boone, J.S., Rice, J. and Glassmeyer, S.T., 2018. Modeled de facto reuse and contaminants of emerging concern in drinking water source waters. Journal‐American Water Works Association, 110(4), pp.E2-E18.
- Khan, S.J., Fisher, R. and Roser, D.J., 2019. Potable reuse: which chemicals to be concerned about. Current Opinion in Environmental Science & Health, 7, pp.76-82.
- Oliveira, T.S., Murphy, M., Mendola, N., Wong, V., Carlson, D. and Waring, L., 2015. Characterization of pharmaceuticals and personal care products in hospital effluent and wastewater influent/effluent by direct-injection LC-MS-MS. Science of The Total Environment, 518, pp.459-478.
- Kwon, M., Royce, A., Gong, Y., Ishida, K.P. and Stefan, M.I., 2020. UV/chlorine vs. UV/H2O2 for water reuse at Orange County Water District, CA: a pilot study. Environmental Science: Water Research & Technology, 6(9), pp.2416-2431.
- Chowdhury, P., Sarathy, S.R., Das, S., Li, J., Ray, A.K. and Ray, M.B., 2020. Direct UV photolysis of pharmaceutical compounds: Determination of pH-dependent quantum yield and full-scale performance. Chemical Engineering Journal, 380, pp.122460.
- Fujioka, T., Khan, S.J., McDonald, J.A., Roux, A., Poussade, Y., Drewes, J.E. and Nghiem, L.D., 2013. N-nitrosamine rejection by nanofiltration and reverse osmosis membranes: The importance of membrane characteristics. Desalination, 316, pp.67-75.
- US Environmental Protection Agency (EPA) 2006. Ultraviolet disinfection guidance manual. Washington, DC: Office of Water.
- Lian, J., Qiang, Z., Li, M., Bolton, J.R. and Qu, J., 2015. UV photolysis kinetics of sulfonamides in aqueous solution based on optimized fluence quantification. Water Research, 75, pp.43-50.